Quantum Cascade Lasers
A wide range of industries, including biomedical, defense, and sensing, utilize tunable mid-infrared lasers that, until recently, have required some rather exotic system configurations. These have historically ranged from free-electron lasers, which require a particle accelerator, to multistage Raman shifted alexandrite lasers, requiring an oscillator-amplifier configuration followed by both deuterium and hydrogen high-pressure gas cells.1,2 Fortunately, over the past twenty years quantum cascade laser (QCL) technology has matured into the laser of choice for long-wavelength operation. To date, lasing wavelengths have been demonstrated from as short as 2.63μm, as long as 250μm under standard operating conditions, and over 300μm with magnetic-field assistance, but most commercially available QCLs tend to be in the 4-11μm range.3,4,5 It should also be noted that less popular QCLs are also commercially available in the terahertz range, up to roughly 150μm.
![Figure 1: Demonstrated QCL emission wavelength/frequency plotted versus operating temperature as of 2015 [6].](/media/kbznjl2n/figure1.jpg?rmode=max)
Figure 1: Demonstrated QCL emission wavelength/frequency plotted versus operating temperature as of 2015.6
Fundamental Operating Principles
The QCL, which was first demonstrated in 1994 at Bell Labs in Murray Hill, NJ, is a semiconductor laser, but it operates under very different physical principles than traditional semiconductor diode lasers.7 Diode lasers achieve stimulated emission through electron-hole recombination between the conduction and valance band. As a result, the emission wavelength is dependent upon the bandgap energy of the particular material. For example, GaAs has a bandgap energy of 1.43 eV, which corresponds to an emission wavelength of 873nm. In contrast, GaN has a much larger bandgap of 3.39 eV, corresponding to a 366nm emission wavelength.8
While the exact emission wavelength can be tuned slightly with temperature and the injection current, the primary method diode fabricators have for varying the emission spectrum is through the use of ternary and quaternary compounds. By changing the stoichiometry of the compounds, one can then tune the bandgap to be anywhere between the bandgaps of the original binary compounds, as long as a lattice matching is maintained.9 Another less common methodology for controlling a diode’s emission wavelength is through a variant known as a quantum well laser (QWL). In these devices a thin layer (< 100Å) of low bandgap material, known as the “well”, is grown between two higher bandgap materials, known as the “barrier” or the “cladding”.10,11 QWLs are primarily utilized for their lower threshold currents, however because of the extremely small size of the well, quantum effects take over, resulting in emission wavelength shifts as a function of well thickness.12
In a QCL, these quantum effects are taken to an extreme by utilizing tens or even hundreds of quantum wells to decouple the emission wavelength from the bandgap energy entirely. The decoupling results from the formation of subbands in the conduction band, allowing for stimulated emission to occur within the conduction band itself, this is known as an intersubband transition.13 Under these circumstances, stimulated emission does not result in electron-hole recombination, leaving the electron within the conduction band. Figure 2 shows a simplified comparison of both interband (conduction-to-valance) and intersubband (conduction-to-conduction) transitions. When combined with a sloped potential bias from well to well, a single electron can cascade through the quantum well structure and emit multiple photons, as shown in Figure 3 below. As a result, QCLs can produce high power at long wavelengths through the combination of both quantum and cascade effects.
Figure 2: Comparison of (a) interband and (b) intersubband transitions in a quantum well laser.
Figure 3:Schematic representation of the cascade effect due to biased quantum well in conjunction with intersubband transitions within a QCL.
Basic Material Structure
In a QCL, the region in which the stimulated emission (intersubband transition) is taking place, is called the active region. The region in which the electron tunnels from the lower energy level of one active region to the upper energy level of the next active region is called the injector region. It is important to note that, unlike in the simplified schematic shown in Figure 3 above, both the active region and the injector region are each constructed of multiple quantum wells. The use of multiple quantum wells is what allows band-structure engineering to control not only the emission wavelength in the active region, but also the electron tunneling energy in the injector region.9 It should also be noted that in the literature, QCLs are often divided into two categories: superlattice QCLs and quantum well QCLs. The main difference between the two categories is that when the quantum wells are thin enough, they no longer form discrete energy levels, but instead blur out to create minibands.14 This naming convention can be a bit misleading because in both cases the material structure is based on a large number of induvial quantum wells, as described previously. Additionally, in all modern QCLs (superlattice and quantum well), the injector region utilizes a single miniband to control the electron tunneling energy so that it lines up with the upper subband of the subsequent active region.15 Figure 4 below shows a schematic representation comparing superlattice and quantum well structures. From this diagram, it is clear that the main difference between the two laser structures is that a quantum well QCL functions as a 4-level laser, whereas a superlattice QCL functions more like a 3-level laser.9
Figure 4:Schematic of quantum well (top) and superlattice (bottom) QCLs, illustrating their respective 4-level and 3-level pumping schemes.
Spectral Properties
QCLs are so well suited to long-wavelength operation because the energy difference between the resultant energy levels in the conduction band is so much smaller than the bandgap energy. This is also the reason why they do not currently operate at shorter wavelengths. The broad gain bands inherent to the miniband structure of superlattice QCLs provide a great deal of flexibility since, at long wavelengths, a small change in energy can result in a substantial shift in wavelength. With this understanding of the fundamental operating principles of QCLs, it is now possible to take a deeper dive into the spectral characteristics of the QCLs on the market today.
Unlike in most laser systems, where temperature tuning is a result of changes to the energy levels associated with the vibrational bands (Boltzmann distribution) of the molecules, QCLs tune through physical expansion and contraction. As the temperature of the QCL is ramped up or down, it will induce slight alterations in the quantum well thickness, resulting in changes to the subband and miniband energy levels. Without extremely tight control over the laser temperature and injection current, this can lead to spectral instabilities and multiple longitudinal modes. For some high-power applications, which will be discussed later, this may be acceptable but for spectroscopic applications, it is not. Therefore, just as with many traditional diode lasers which also have broad gain bands, a grating can be utilized as both wavelength stability and tunability. In fact, by 1997, less than three years after the first QCL was demonstrated, the team at Bell Labs fabricated the earliest distributed feedback (DFB) QCL.16 Like any other DFB laser, DFB-QCLs utilizes Bragg grating, which is etched into the semiconductor to act as a wavelength-selecting device. These devices can then be temperature-tuned while maintaining single wavelength operation. While compact and practical, the overall tunability range of a DFB-QCL is typically limited to 10-20 cm-1.17
For larger tuning ranges, an external cavity (EC) design is far more effective. In an EC-QCL the front facet of the semiconductor is coated to significantly reduced the reflectivity, therefore increasing the gain threshold. Next, a grating is used as an output coupler, resulting in a wavelength selective decrease in the gain threshold therefore restricting lasing to a single frequency. By tuning the grating, typically trough rotation, an EC-QCL can then be wavelength-tuned over the entire gain band of the QCL, which has been demonstrated to provide tunability ranges of over 400 cm-1.18 It should be noted that, while DFB-QCLs require single-spatial mode (TEM00) operation, EC-QCLs do not which can allow for higher power operation. Whenever an EC-QCL is not built with a single-spatial mode QCL it can never be considered single-frequency, it is merely wavelength stabilized.
Common Applications
With this understanding of the basic functionality and spectral characteristics associated with QCLs, it is now possible to understand why they have become an invaluable tool for many applications. While there are countless applications in which QCLs have been utilized over the years, the three applications where QCLs have gained the most traction are absorption spectroscopy, trace-gas detection, and infrared countermeasures. Therefore, the remainder of this article will focus on how QCLs are utilized in each of these three application spaces.
Absorption Spectroscopy
Of the primary three applications for QCLs, in many respects absorption spectroscopy has been the most prolific. All covalently bound materials have a unique absorption spectrum in the infrared spectrum due to the allowed vibration and rotation modes arising from the molecular structure.9 Since no two materials will have the same molecular structure, the various absorption bands associated with each bond form a unique spectrum, known as a molecular fingerprint. Traditionally, infrared absorption is measured using a Fourier transform infrared (FT-IR) spectrometer. These devices consist of a broadband infrared light source (known as a globar), and a scanning interferometer, which is internally calibrated using a stabilized helium-neon laser. QCLs substantially simplify the system by completely removing interferometer from the set-up and replacing the infrared light source with a tunable QCL. As a result, QCLs are now widely utilized in chemical analysis and chemical imaging because they can significantly reduce the cost and complexity of the system. It is also important to mention that absorption spectroscopy can also be performed in the terahertz region as well, where QCLs are readily available.
Trace-Gas Detection
While technically a subset of absorption spectroscopy, trace-gas detection has matured into a classification of its own. This is not only because of the infrared absorption of the gas analytes but also because of the atmospheric transmission bands also line up nicely in the mid-infrared as well, as shown in Figure 5. Figure 5 also highlights the tunability range of several commercially available DFB-QCLs.19 There is a wide range of measurement methodologies used for trace gas detection in the infrared, but the primary technique being utilized today is tunable diode laser absorption spectroscopy (TDLAS). In TDLAS, a laser is rapidly tuned over a single line in the infrared absorption spectrum of the analyte to map the intensity and shape of the cross-section. By scanning a single-frequency laser source across the absorption band, the entire line-shape function of the vibrational band can be characterized, allowing for the exact molecular concentration to be calculated. Even though the acronym TDLAS includes the word diode, QCLs are more commonly used for these applications because of the necessary wavelengths and tuning ranges. Additionally, it should be noted that while many of these gasses also have corresponding electronic absorption bands in the ultraviolet and visible, Rayleigh scattering at those wavelengths makes them improbable for remote sensing applications.
![Figure 5: The atmospheric transmission spectrum, along with the tuning range of several DFB-QCLs at critical gas absorption bands [19].](/media/vbxpplxm/figure5.png?rmode=max)
Figure 5: The atmospheric transmission spectrum, along with the tuning range of several DFB-QCLs at critical gas absorption bands.19
Infrared Counter Measures
Since the early days of heat-seeking missile technology, engineers have been developing countermeasures intended to disorient the infrared guidance sensors. Initially consisting of flares that the pilot could deploy when under attack, the technology quickly evolved into reusable heat sources, similar to the globar used in modern FT-IR spectrometers. In addition to being reusable, these devices had the added benefit that they could remain active during the entire mission. In 1997, shortly after the invention of the QCL, the United States Navy patented one of the earliest known laser infrared countermeasures.20 The patent explicitly states that the optimal wavelengths for the two lasers in the lasers should be at ~4μm and ~5μm to correspond to the operating wavelengths of modern infrared guidance sensors. All heat-seeking missiles operate in this range because they correspond to atmospheric windows, shown in Figure 5, and they are also short enough to avoid interference from terrestrial blackbody radiation (at around 10μm) while also long enough to prevent interference from solar radiation in the near-infrared. Since QCLs are the only truly lightweight, efficient, and compact laser source in that region, they have become the laser of choice for infrared countermeasures. Unlike the previous two applications, which require single frequency QCLs, infrared countermeasures utilize high power multimode QCLs.
Final Thoughts
Over the next twenty years, there is no doubt that QCLs will continue to become more commonplace as the technology matures and the cost continues to decrease. As this technology becomes more and more prevalent, scientists and engineers will continue to new and exciting applications. Here at Edmund Optics®, we are committed to supplying the community with the highest-quality Infrared Optics to help facilitate these advancements. For example, we offer a line of Germanium Aspheric Lenses, designed to operate from 3μm to 50μm as well as a full suite of Off-Axis Parabolic and Ellipsoidal Mirrors.
References
- Edwards, G.S., Austin, R.H., Carroll, F.E., Copeland, M.L., Couprie, M.E., Gabella, W.E., Haglund, R.F., Hooper, B.A., Hutson, M.S., Jansen, E.D. and Joos, K.M., 2003. Free-electron-laser-based biophysical and biomedical instrumentation. Review of scientific instruments, 74(7), pp.3207-3245.
- Kozub, J., Ivanov, B., Jayasinghe, A., Prasad, R., Shen, J., Klosner, M., Heller, D., Mendenhall, M., Piston, D.W., Joos, K. and Hutson, M.S., 2011. Raman-shifted alexandrite laser for soft tissue ablation in the 6-to 7-μm wavelength range. Biomedical optics express, 2(5), pp.1275-1281.
- Cathabard, O., Teissier, R., Devenson, J., Moreno, J.C. and Baranov, A.N., 2010. Quantum cascade lasers emitting near 2.6μm. Applied Physics Letters, 96(14), p.141110.
- Walther, C., Fischer, M., Scalari, G., Terazzi, R., Hoyler, N. and Faist, J., 2007. Quantum cascade lasers operating from 1.2 to 1.6 THz. Applied Physics Letters, 91(13), p.131122.
- Wade, A., Fedorov, G., Smirnov, D., Kumar, S., Williams, B.S., Hu, Q. and Reno, J.L., 2009. Magnetic-field-assisted terahertz quantum cascade laser operating up to 225 K. Nature Photonics, 3(1), pp.41-45.
- Vitiello, M.S., Scalari, G., Williams, B. and De Natale, P., 2015. Quantum cascade lasers: 20 years of challenges. Optics express, 23(4), pp.5167-5182.
- Faist, J., Capasso, F., Sivco, D.L., Sirtori, C., Hutchinson, A.L. and Cho, A.Y., 1994. Quantum cascade laser. Science, 264(5158), pp.553-556.
- Fukuda, M., 1998. Optical semiconductor devices (Vol. 46). John Wiley & Sons.
- Saleh, B.E. and Teich, M.C., 2019. Fundamentals of photonics. john Wiley & sons.
- Nag, B.R., 2001. Physics of quantum well devices (Vol. 7). Springer Science & Business Media.
- Odoh, E.O. and Njapba, A.S., 2015. A review of semiconductor quantum well devices. Adv. Phys. Theor. Appl, 46, pp.26-32.
- Agullo-Lopez, F. and Cabrera, J.M., 1994. Electrooptics: phenomena, materials and applications (Vol. 1). Academic Press.
- Helm, M., 1999. The basic physics of intersubband transitions. In Semiconductors and semimetals (Vol. 62, pp. 1-99). Elsevier.
- Sirtori, C. and Teissier, R., 2006. Quantum cascade lasers: overview of basic principles of operation and state of the art. Intersubband Transitions in Quantum Structures, pp.1-64.
- Pecharromán-Gallego, R., 2017. An Overview on Quantum Cascade Lasers: Origins and Development. In Quantum Cascade Lasers. IntechOpen.
- Faist, J., Gmachl, C., Capasso, F., Sirtori, C., Sivco, D.L., Baillargeon, J.N. and Cho, A.Y., 1997. Distributed feedback quantum cascade lasers. Applied Physics Letters, 70(20), pp.2670-2672.
- Hugi, A., Maulini, R. and Faist, J., 2010. External cavity quantum cascade laser. Semiconductor Science and Technology, 25(8), p.083001.
- Hugi, A., Terazzi, R., Bonetti, Y., Wittmann, A., Fischer, M., Beck, M., Faist, J. and Gini, E., 2009. External cavity quantum cascade laser tunable from 7.6 to 11.4μm. Applied Physics Letters, 95(6), p.061103.
- Gmachl, C., Capasso, F., Sivco, D.L. and Cho, A.Y., 2001. Recent progress in quantum cascade lasers and applications. Reports on progress in physics, 64(11), p.1533.<
- Meeker, D.B., US Secretary of Navy, 1997. Infrared projector countermeasure system. U.S. Patent 5,703,314.
Related Edmund Optics Products
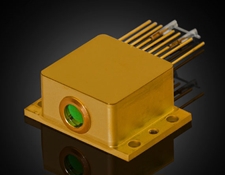
Quantum Cascade Laser (QCL) Systems
- CO2, N2O, and NO Tuned Quantum Cascade Laser Systems
- Laser, Power Supply, Detector, and Control Electronics Included
- Ideal for Absorption Spectroscopy and Trace Gas Detection

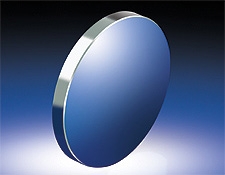
Germanium Infrared (IR) Aspheric Lenses
- Diffraction Limited Performance
- Variety of Coating Options
- Full Prescription Data Available

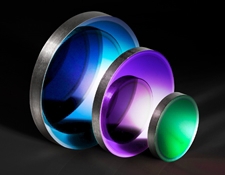
- AR Coating Options for a Variety of IR Ranges
- Minimal Chromatic Aberration Due to Low Dispersion
- Ideal for Infrared Applications Requiring Rugged Optics

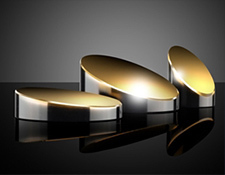
Off-Axis Parabolic and Ellipsoidal Mirrors
- AR Coating Options for a Variety of IR Ranges
- Minimal Chromatic Aberration Due to Low Dispersion
- Ideal for Infrared Applications Requiring Rugged Optics

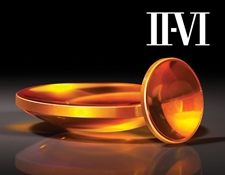
Zinc Selenide (ZnSe) Aspheric Lenses
- Edmund Optics Designed, II-VI Manufactured
- Premier Grade ZnSe Material
- Uncoated or Broadband 8-12μm AR Coating Available

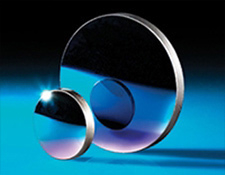
View All Edmund Optics Infrared Optics
- Wide Variety of Substrates and Coatings
- Ideal for Precise Temperature Monitorin
- Large Global Inventory
More Resources
- Beam Quality and Strehl Ratio Application Note
- Gaussian Beam Propagation Application Note
- Laser Resonator Modes Application Note
- Laser Optics Lab Video Series
- Simplifying Laser Alignment Application Note
or view regional numbers
QUOTE TOOL
enter stock numbers to begin
Copyright 2023, Edmund Optics Inc., 101 East Gloucester Pike, Barrington, NJ 08007-1380 USA
California Consumer Privacy Acts (CCPA): Do Not Sell or Share My Personal Information
California Transparency in Supply Chains Act